OR/14/048 Results of petrographical analyses
Rochelle, C A, Purser, G, and Milodowski, A E. 2014. Results of laboratory carbonation experiments on NRVB cement. British Geological Survey Internal Report, OR/14/048. |
Unreacted starting cement
The cured, but unreacted, NRVB cement cores used in the batch experiments were characteristically mid-to-light grey in colour (Plate 1A). Petrographic analysis shows that several (though by no means all) of the NRVB cement plugs used were significantly heterogeneous. The surfaces of the cement cores often displayed a ‘marbled’ or ‘wood-grained’ effect, which in thin section was seen to be due to fine lamination in the cement (Plate 1B). This lamination results from particle-size segregation of the cement paste into finer- and coarser-grained layers. The segregation is clearly a primary feature of the hydrated cement produced by a combination of one or more of following factors:
- Incomplete or poor mixing during preparation of the cement paste. In this context it should be noted that the NRVB cement paste used in these experiments was prepared using only a basic portable builders cement mixer, rather than a high-shear cement blender system;
- Hydrodynamic flow-separation of cement paste on the basis of the difference in density and grain size differences of components within the cement paste, during pouring the cement into plastic moulds used for casting the blocks of cement, from which the core plugs were subsequently cut. In this context, it should be noted that no special additives were used that might prevent segregation of the NRVB cement components;
- Hydrodynamic segregation of material on the basis differences in particle size and density, as a result of settling and dewatering of the cement after pouring the cement paste into the plastic moulds. In places, the laminae are contorted and disturbed, and closely resembling soft-sediment deformation structures formed by dewatering of unconsolidated sediments (e.g. Allen, 1982[1]; Mills, 1983[2]), and suggests some gravitational settling and dewatering of the cement paste has occurred immediately after pouring the cement paste and before cement setting and hardening. Further evidence of post-pouring segregation of the cement can be found in the development of a thin layer of very fine cement paste, which appears to have settled out on the upper surface of some of the unreacted cement core sample. In this context, it should be noted that no special additives were used during cement preparation to inhibit any physical segregation occurring within the cement paste. It is possible therefore, that without additives such segregation features may occur within actual repository cement.
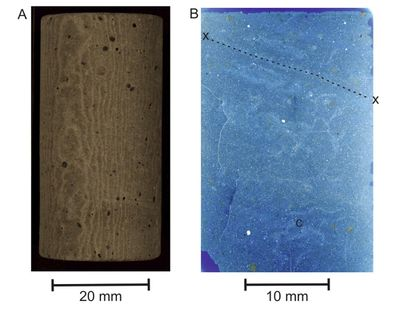
Small (0.1 to 2 mm diameter) dark grey particles of dense micritic limestone aggregate were visible in the core plug surface (Plate 1A) and were also apparent in thin section. The cement cores also contained entrained air bubbles that had been incorporated into the cement paste during mixing. These represent macropores ranging in size from 0.2 to 1 mm diameter and are commonly partly filled or lined by euhedral, hexagonal plates of portlandite (Ca(OH)2) (Plate 2). In the outer surface of the cement, the portlandite crystals are etched and corroded (Plate 3), and the corroded surfaces are partly encrusted with very fine secondary calcium carbonate crystals. Some of the calcium carbonate exhibits rhombohedral morphology and is, therefore, most probably calcite. This indicates that the outer surfaces of the cement had already reacted to a small degree with CO2 prior to the batch experiment run. This CO2 is likely to have been derived from direct exposure atmospheric CO2 during core handling or by diffusion of atmospheric CO2 into the storage containers prior to experimentation. Portlandite found lining entrained air bubbles in the centre of the cement plug generally had pristine crystal faces and showed no alteration.
-
Plate 2 SEM (SEI) photomicrograph of an air bubble in NRVB cement paste. The cavity is partially filled with hexagonal plate-like crystals of portlandite (Ca(OH)2). Unreacted NRVB cement paste starting material. -
Plate 3 SEM (SEI) photomicrograph showing dissolution and corrosion of a portlandite crystal formed within an air bubble exposed in the outer surface of the NRVB cement core. The surfaces of the corroded portlandite crystal are coated with very fine secondary calcium carbonate. Unreacted NRVB cement paste starting material.
The cement comprises fragments of fine silt to fine sand-grade limestone aggregate, within a very fine amorphous or nanocrystalline gel-like calcium silicate hydrate (CSH) matrix (Plate 4). Within this, remanant fragments of primary cement clinker grains are present. These consist of relatively unaltered calcium aluminate-ferrite (CAF) phases, probably brownmillerite (Ca2(Al,Fe)2O5), intergrown with amorphous of microcrystalline CSH, hydrated calcium aluminate (CAH) and hydrated calcium aluminosilicate (CASH) hydration products (replacing the original primary anhydrous calcium silicates and aluminosilicates) (Plate 4).
Detailed observations of the laminated fabric observed in the cement reveal marked mineralogical and fabric differences between the laminations. The lighter and coarser laminae observed in thin section (Plate 1B), have a higher proportion of coarser silt-grade limestone aggregate particles (Plate 5). They also contain patches of relatively coarsely-crystalline portlandite poikilotopically-cementing the intergranular microporosity, and which also displaces the gel-like CSH-rich interstitial matrix within these coarser bands (Plate 5). These patches may coalesce to form relatively continuous bands at the thin section scale. In contrast, the darker finer laminations (Plate 1B) have a higher proportion of finer limestone aggregate particles and much less (and finer scaled) interstitial/intergranular portandite development.
-
Plate 4 BSEM photomicrograph of showing a section through the outer edge of the cement plug (left edge of image). A thin dense layer of microcrystalline calcite (light grey) can be seen to form a continuous outer layer, with finely-disseminated calcite (light grey) permeating the CASH gel matrix (dark grey) of the cement. Coarse fragments of limestone aggregate can be seen as the relatively large grains of calcium carbonate (light grey). Remnants of primary cement clinker grains are present, with relatively unaltered brownmillerite (bright grains) intergrown with CSH hydration products (dull grey) replacing the original primary anhydrous calcium silicate. Immediately beneath the dense outer calcium carbonate skin, the cement has been dissolved with interconnected dissolution microporosity (black) Unreacted NRVB cement paste starting material. -
Plate 5 BSEM photomicrograph of showing patches of relatively coarsely-crystalline portlandite crystals (light grey) cementing the microporosity and enclosing silt-grade limestone aggregate particles, and growing displacively within the CSH-rich gel matrix. The image is characteristic of the apparently coarser and lighter laminae seen in thin section under transmitted light (Plate 1B). Unreacted NRVB cement paste starting material.
The petrographic observations show that this laminated fabric has developed as a result of physical separation of the finer and coarser particle sizes of the cement clinker and limestone flour aggregate. This clearly occurred as through hydrodynamic particle-size fractionation during manufacture of the stock NRVB cement starting material from which the cement cores were cut. This process appears to have produced a heterogeneous laminated fabric with coarser and pore porous (and presumably more permeable) laminae within which portandite precipitated in the intergranular porosity from the cement porewater during sample curing. Whereas, the finer and less permeable laminae developed much less portlandite during the curing process.
The segregation of the cement past into fine and coarse laminae influences the porosity distribution in the cement, and thereby will affect the permeability of gas flow pathways through the cement cores. Observation of the blue-dye epoxy-resin impregnation of the thin sections by transmitted-light optical microscopy suggests that the coarser laminae display greater degree of resin impregnation, and hence greater porosity than the finer laminae. This heterogeneity in the primary cement fabric, produced during the manufacture of the cement core samples, was observed to have had a significant influence on the pattern and extent of alteration and CO2 diffusion pathways in several of the cement cores during the carbonation of the cement in the batch experiments.
BSEM-EDXA observations of the polished thin section profile from the rim to centre of the unaltered cement revealed that a thin layer of carbonated cement was already present on the external surfaces of the cement cores before the carbonation experiments started (Plate 4). The carbonated cement layer formed a near-continuous skin over the surface of the cement plug (Plate 4). It consisted of a dense outer layer of tightly-interlocking microcrystalline calcium carbonate up to 50 μm thick, within which the original hydrated cement phases have been virtually completely replaced by calcium carbonate. This indicates that there has been almost complete migration and loss of Si and Al from within this narrow alteration zone. Beneath this, a band of more pervasive impregnation and partial replacement of the underlying CSH matrix by fine-grained calcium carbonate extends for up to 150 μm from the surface of the cement. Immediately beneath the dense outer carbonated layer, the cement shows evidence of dissolution of the CASH and CAH/CASH phases, with subsequent enhanced porosity, extending over a zone up to 50 μm wide. Coarse clear colourless or white crystals up to 2 mm long were observed sporadically on the surfaces of the unfreacted cores after curing (Plate 6). SEM-EDXA showed that these were also calcium carbonate. This mineralogical alteration indicated that the cement had already undergone some reaction with atmospheric CO2 and dissolution in the water during the curing and storage of the cement plugs prior to use in the experiments.
Analyses of experimentally carbonated cement
The following sections describe observations made of different parts of the cement blocks after they had undergone carbonation tests.
Surface alteration characteristics of the cement cores
Upon reaction with CO2 (gaseous, liquid or supercritical) most cement blocks showed a marked colour change from grey to brown (Plate 6). Petrographic analysis shows that this colour change results from the reaction of calcium ferrite or calcium aluminoferrite phases (e.g. brownmillerite) with CO2 to produce secondary calcium carbonates accompanied by the liberation of finely-disseminated free ferric oxide (Fe2O3) or ferric oxhydroxide (Fe2O3.nH2O) to give a ‘rusty’ colour.
- e.g. [CaO]2.Fe2O3 + 2 CO2 ⇒ 2 CaCO3 + Fe2O3
These preliminary observations are consistent with results from studies of CO2 reaction with borehole cement as part of studies into the deep underground storage of CO2 (e.g. Carey et al., 2007[3]; Rochelle et al., 2007[4]). It is possible therefore, that similar CO2-cement mineral reactions are taking place. Should further analyses prove this to be the case, then there could potentially be useful exchange of data and modelling approaches between radioactive waste studies and carbon capture and storage studies.
All of the reacted cement cores exhibited extensive precipitation of secondary calcium carbonate reaction products on their external surfaces. The morphology of the carbonate varied considerably, from: dense crystalline calcite and aragonite encrustations with dry supercritical CO2 (e.g. Plate 7), with supercritical CO2 and YNFP (e.g. Plate 8), and supercritical CO2 and ENFG; to encrustations of loosely-adhering microcrystalline sub-rounded rhombohedral calcite and rare coarse calcite rhombs after reaction with supercritical CO2 and ENFG (e.g. Plate 9); to microporous bladed orthorhombic crystal aggregates of probable aragonite with dry liquid CO2 (e.g. Plate 10); and fine tightly interlocking ‘scaley’ microcrystalline calcite and gelatinous (possibly amorphous) calcium carbonate seen with liquid CO2 and ENFG (e.g. Plate 11).
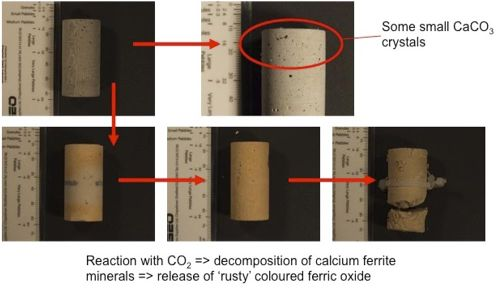
-
Plate 7 SEM (SEI) photomicrograph of the surface of cement plug after reaction with dry supercritical CO2 after 40 days at 40°C and 80 bar. The surface is encrusted with tightly interlocking crystals of trigonal-rhombohedral calcite (lower half of image) and spheroidal radial-fibrous aggregates of acicular, bladed or elongated prismatic calcium carbonate (upper half of image) that are probably aragonite. -
Plate 8 SEM (SEI) photomicrograph of the surface of cement plug after reaction with YNFP and supercritical CO2 after 40 days at 40°C and 80 bar. The surface is encrusted with tightly-interlocking crystals of calcite.
-
Plate 9 SEM (SEI) photomicrograph of the surface of cement plug after reaction with ENFG and supercritical CO2 after 40 days at 40°C and 80 bar. The surface is encrusted with loosely-adhering, finely crystalline equant sub-rounded rhombohedral crystals of calcite. -
Plate 10 SEM (SEI) photomicrograph of the surface of cement plug after reaction with dry liquid CO2 after 40 days at 20°C and 80 bar, showing encrustation of the surface by aggregates of elongated to bladed orthorhombic prisms of possible aragonite crystals.
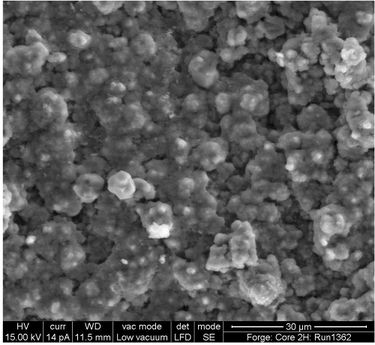
Calcium carbonate precipitation on the cement surfaces was observed in reacted cores from both CO2 and N2 control experiments. No consistent relationship was found between the morphology of the calcium carbonate coating the external surfaces of the cement cores and the experimental conditions. The carbonation observed in the N2 control experiments suggests that either the N2 experimental cells were not entirely free of atmospheric CO2, or that the sealing of the reacted samples within the Ar-flushed crimp-welded flat-roll tubing was nor completely effective in preventing reaction with atmospheric CO2 prior to petrographic analysis.
Carbonation with gaseous CO2
Detailed petrographic analysis was undertaken on the cement cores reacted with YNFP and ENFG in the presence of gaseous CO2 and with dry gaseous CO2, all reacted for 40 days at 40°C under 40 bar pressure. Plate 12 compares transmitted light images of polished thin sections illustrating profiles through reacted cores for experiments with YNFP and ENFG in the presence of gaseous CO2, with dry gaseous CO2, and unreacted cement. The degree and pattern/pathway of alteration was observed to be markedly different between the three experiments ‘YNFP + gaseous CO2’, ‘ENFG + gaseous CO2’ and ‘dry gaseous CO2 only’. The transmitted light photomicrograph of the reacted cement core from experiment with ‘YNFP(1) + CO2’, is also shown in Figure 13. This sample was not examined in detail but appears to show broadly similar alteration characteristics to that in the ‘YNFP+Cl + gaseous CO2’ experiment.
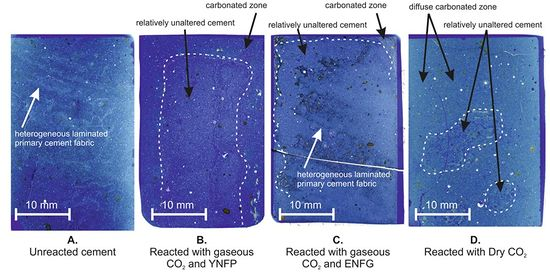
The alteration pattern and pathways taken by the alteration in ‘YNFP+Cl + gaseous CO2’ are strongly influenced by the heterogeneity (particle size laminations and segregations) in the original cement paste, which most probably controlled variation in primary permeability of the cement core.
The ‘YNPF + gaseous CO2’ experiments showed the sharply-defined reaction fronts. Both ‘YNFP+Cl + gaseous CO2’ and ‘YNFP + gaseous CO2’ experiments show broadly similar alteration patterns (Plate 12B and Plate 13). The alteration is characterised by broadly concentric alteration fronts, with an outer zone of intensely carbonated cement up to 6 mm wide and an inner zone of relatively unaltered cement (although detailed BSEM-EDXA observations show at least some carbonation within this inner region of the cores). In the case of the ‘YNFP+Cl + gaseous CO2’ experiment, a 0 to 8 mm wide zone of enhanced porosity (shown in the thin section by greater blue-dye resin impregnation in Figure 13) is seen between the outer carbonated zone and the relict unaltered core of the cement. In the case of the ‘YNFP + gaseous CO2’ experiment, this zone of enhanced porosity is very poorly-developed to imperceptible, and where developed it is confined to an extremely narrow zone less than 250 µm wide and immediately in front of and at the edge of the carbonation front (Plate 14). The reason for this difference observed in the alteration behaviour of these two similar experiments is unclear. However, this may in part be influenced by differences in the heterogeneity of the two cement cores. In constrast to the ‘YNFP + gaseous CO2’ experiment, the cement core in the ‘YNFP+Cl + gaseous CO2’ possessed significant primary heterogeneity due to lamination and particle size segregation in the original cement. This appeared to have influenced at least some of the diffusion pathways for CO2 migration into the cement during the experiment, and may have provided greater access of CO2 to the cement along the more permeable coarser laminations.
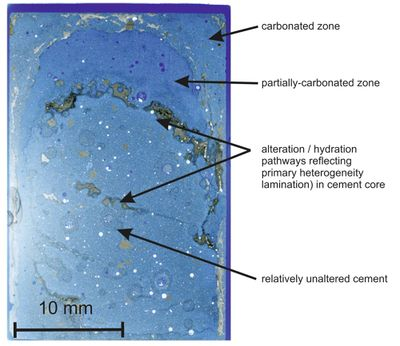
In both ‘YNFP+Cl + gaseous CO2’ and ‘YNFP + gaseous CO2’ experiments, the carbonation of the cement is associated with the development of a series of carbonation reaction fronts, along which a an anastomising network of very fine (5–10 µm wide) microfractures is developed sub-parallel to the alteration front (Plate 14). As this front moves forward the microfractures are mineralised by calcium carbonate to produce a ‘chicken-wire’ meshwork fabric of microfractures within the carbonated cement behind the advancing reaction front. As it moves into the cement core (Plate 14 and Plate 15). Within the carbonated cement, the hydrated CSH and CASH/CAH hydrated cement compounds are progressively altered at the reaction front, to produce a matrix of finely-disseminated micro- to nanocrystalline calcium carbonate dispersed within an amorphous gel-like residual silica rich groundmass (Plate 16).
-
Plate 14 BSEM photomicrograph of the interface between the intensely-carbonated cement (top and left of image) and residual relatively unaltered core (bottom and right of image) of the reacted cement plug from the ‘YNFP + gaseous CO2’, 40 days at 40°C under 40 bar pressure experiment. The carbonated cement zone displays a ‘chicken-wire’ alteration fabric of anastomising interconnected microfractures and shrinkage cracks sealed by microcrystalline calcium carbonate. Entrained air bubbles are mineralised by coarsely crystalline aragonite in the carbonated cement and by plates of portlandite in the unaltered cement. -
Plate 15 BSEM photomicrograph of the carbonated cement zone showing the ‘chicken- wire’ alteration fabric of anastomising interconnected microfractures and shrinkage cracks sealed by microcrystalline calcium carbonate (white), within a very fine matrix of microcrystalline calcite disseminated in a amorphous silica groundmass. ‘YNFP + gaseous CO2’, 40 days at 40°C under 40 bar pressure experiment.
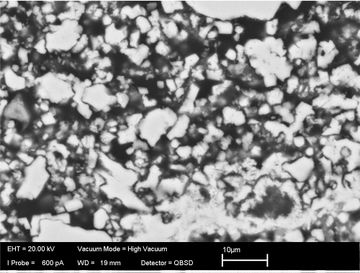
The matrix of the carbonated cement appears to have broadly similar matrix microporosity porosity to that of the unaltered cement. Although, as described above, the porosity may be enhanced locally by dissolution of CSH and CAH/CASH phases immediately ahead of the main carbonation front seen in the ‘YNFP+Cl + gaseous CO2’ experiment. The macroporosity, represented by the entrained air bubbles in the cement past were observed to be unmineralised or partially-filled by hexagonal plates of portlandite in the residual unaltered cement ahead of the carbonation front (Plate 14). However, in the carbonated cement zone the portlandite has been removed and the cavities are now lined or filled by orthorhombic prisms or acicular calcium carbonate (Plate 14), which (on the basis of its morphology) is most probably aragonite.
Although, the carbonation alteration is strongest within the fully-carbonated region of cement behind the sharply-defined carbonation front, it was clear that CO2 had diffused further into the cement cores ahead of the main reaction front. This has resulted in patchy, diffuse alteration, and partial replacement of the hydrated CSH and CASH cement phases thoughout the cement core by very fine (sub-micron) calcium carbonate material.
EDXA showed enhanced concentration of Cl in the matrix of the alterated cement, at the leading edge of the carbonation front. However, unlike in the experiments with ‘ENFG and gaseous CO2’, no discrete Cl-rich phase was found by BSEM-EDXA.
The ‘ENFG + gaseous CO2’ experiment (40 days at 40°C under 40 bar pressure) produced a much narrower and less developed zone of carbonation in the reacted cement plug than in the two ‘YNFP + gaseous CO2’ experiments (Plate 12 and Plate 17). However, the carbonation reaction front is sharply-defined (Plate 17), as was seen in the ‘YNFP + gaseous CO2’ experiments. The carbonated zone varied from only 250 µm to about 1 mm in thickness (Plate 17). This experiment also produced a carbonated alteration zone with similar development of a ‘chicken-wire-like’ network fabric of anastomosing calcium carbonated sealed microfractures to that seen in the YNFP experiments. However, the degree of carbonation and alteration within this very narrow zone was much more intense with more complete replacement of the cement matrix by calcium carbonate (Plate 18) than was observed in the ENFG experiment. The secondary calcium carbonate appeared to be coarser than in the YNFP fluid experiments, and many of the microcrystals displayed rhombohedral form, suggesting that the secondary calcoum carbonate phase is dominantly calcite rather than the aragonite or vaterite polymorphs.The ‘ENFG + gaseous CO2’ experiment also displayed more complicated alteration at the carbonation reaction front than was observed in the ‘YNFP + gaseous CO2’ experiments. Detailed BSEM-EDXA observations revealed the formation of secondary calcium chloroaluminate alteration products at or immediately behind the leading edge of the carbonation front in this experiment (Plate 19 and Plate 20). A similar secondary calcium chloroaluminate alteration product was observed by Rochelle et al. (2009)[5], in experiments on the interaction of CO2 with Portland cement-based oilwell ‘Type G’ cement. XRD analysis of the altered oilwell cement tentatively identified the crystalline product observed previously by Rochelle et al. (2009)[5] as hydrocalumite (Ca4Al2O6Cl2.10H2O). This phase has nucleated within, and is enclosed by, a matrix of amorphous silica-rich gel and finely-disseminated calcium carbonate (Plate 20).
-
Plate 17 BSEM photomicrograph of the interface between the intensely-carbonated cement (brighter region at the top left of the image) and residual relatively unaltered core (predominantly duller region at the bottom and right of image) of the reacted cement plug from the ‘ENFG + gaseous CO2’, 40 days at 40°C under 40 bar pressure experiment. -
Plate 18 BSEM photomicrograph of the intensely-carbonated cement from the ‘ENFG + gaseous CO2’, 40 days at 40°C under 40 bar pressure experiment, showing extensive replacement of the original CSH and CAH/CASH matrix by microcrystalline rhombs of calcite. The image also shows part of a tight, calcite-filled microfracture from close to the interface between altered and unaltered cement (bottom left of image).
-
Plate 19 BSEM photomicrograph of the carbonation reaction front between intensely-carbonated cement (top left) and residual unaltered cement (bottom right) from the ‘ENFG + gaseous CO2’, 40 days at 40°C under 40 bar pressure experiment. Dense microcrystalline calcium carbonate is developed at the leading edge of the front and a radial fibrous calcium chloroaluminate phase has formed within altered cement immediately behind the front. -
Plate 20 BSEM photomicrograph showing detail of the radial fibrous secondary calcium chloroaluminate phase formed within altered cement immediately behind the leading edge of the carbonation front. The BSEM brightness increases towards the outer edge of the phase as Fe:Al increases. The calcium chloroaluminate phase is enclosed in a matrix of Si-rich gel (dull grey) with finely-disseminated calcium carbonate (light grey) ‘ENFG + gaseous CO2’, 40 days at 40°C under 40 bar pressure experiment.
Detailed BSEM-EDXA petrographic analyses show that this reaction product is strongly growth-zoned (Plate 20, Figure 14). The early growth stages of the radial-fibrous crystals are Al-rich with only minor Fe (Figure 14). However, as growth proceeds, the crystals become increasingly enriched in Fe with a corresponding decrease in Al (Figure 14). This demonstrates that there is significant solid-solution, with Fe substitution for Al within this calcium chloroaluminate phase, and that Fe is being mobilised and enriched in the cement pore fluids with time as alteration at the leading edge of the carbonation front progresses.
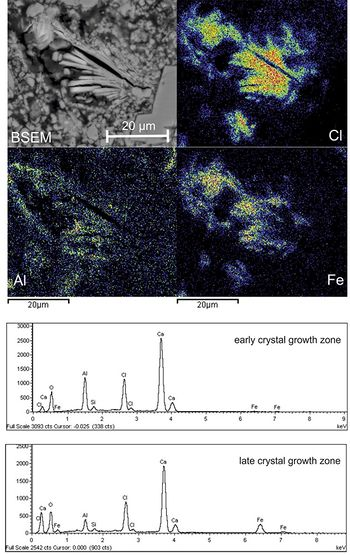
The formation of this secondary calcium chloroaluminate is responsible for a concentration of chloride at or just behind the leading edge of the main carbonation front in these experiments with ‘ENFG + gaseous CO2’. However, this phase is transient and is absent in the fully-carbonated cement. As the carbonation front moves forward the calcium chloraluminate phase is dissolved and replaced by calcium carbonate and fine grained aluminous material. The chlorine then continues to migrate further into the cement, following the carbonation front, to reform and precipitate fresh crystals of calcium chloroaluminate
The precipitation of calcium chloroaluminate as a reaction product in these experiments accounts for the significant depletion of Cl- observed in the fluid. Although only a transient phase, the formation of this reaction product may potentially be important as a mechanism of retarding the migration of 36Cl from low- and intermediate-level radioactive waste.
Although Cl was observed to be lost from the fluid phase during the course of the control experiments with gaseous N2, no petrographic evidence of Cl concentration or secondary Cl-rich alteration products were observed. EDXA maps for Cl showed uniformly-distributed low concentration of Cl throughout the cement in the control experiments with gaseous N2.
As seen in the ‘YNFP + gaseous CO2’ experiments (see earlier), although the carbonation alteration is strongest within the fully-carbonated region of cement left in the ‘wake’ of the sharply-defined advancing carbonation front, diffuse alteration by CO2 was observed to have penetrated deeper into cement cores ahead of the main reaction front. This, again resulted in patchy, diffuse and partial replacement of the hydrated CSH and CASH cement phases by very fine (sub-micron) calcium carbonate material.
The cement core that had been reacted with ‘Dry CO2’ at 40° C displayed a marked colour change from initially grey cement to orange-brown stained cement (Plate 21). The alteration fabric is markedly different to that observed with either ‘YNFP + gaseous CO2’ or ‘ENFG + gaseous CO2’. The cement from this sample appears to have been carbonated through much of the volume of the core, with only a small proportion of relatively unaltered cement remaining near the middle of core plug (Plate 12). The carbonation alteration is pervasive and diffuse, and extremely fine grained, and the altered cement has a nano-to-microporous matrix. The strong reaction fronts, with relatively coarse and tightly-interlocking crystalline calcite (or other calcium carbonates) observed in the ‘YNFP + gaseous CO2’ or ‘ENFG + gaseous CO2’ experiments were not developed in this experiment with dry CO2.
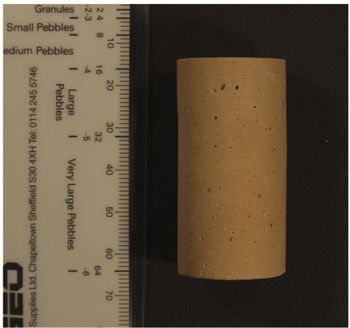
Carbonation with supercritical CO2
The alteration characteristics of NRVB cement with supercritical CO2 in the presence of YNPF or ENFG are very similar. As with gaseous CO2 (see Surface alteration characteristics of the cement cores), the reaction with CO2 is demarcated by very sharp reaction fronts (Plate 21), which migrate deeper into the cement with time. In the case of the ‘YNPF+Cl + supercritical CO2’ experiment, the cement sample appears to be completely carbonated after 1 year (Plate 22). However, in the experiments with ENFG the carbonation fronts appear to advance more lowly than with YNPF, and even after a year of reaction, a relict core of only partially carbonated cement is still present (Plate 22). Transmitted light optical microscope observations show that there is an intermediate zone of of slightly more nano- to micro-porous cement developed ahead of the main carbonation front. This appears to be partially altered to very fine grained calcium carbonate but also partially leached of Ca in comparison to either the dense calcite-replaced outer reaction zone or the residual and partially altered cement in the core of the cement plugs.
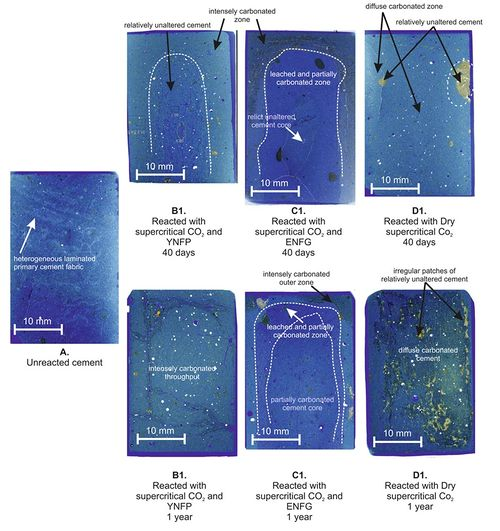
The alteration by dry supercritical CO2 does not produce discrete reaction fronts. Instead, it resulted in diffuse alteration to a highly-carbonated cement comprising a mixture of very fine grained calcium carbonate and secondary silica-rich material, and with finely disseminated iron oxide is produced (Plate 22). Within this fine-grained highly-carbonated matrix, irregular relicts of unreacted cement paste may be present. These unreacted relicts are heterogeneously distributed, and reflect the original heterogeneous character of the original fabric of the cement paste.
Detailed BSEM-EDXA observations show that the alteration fabric within the outer, and fully-carbonated regions of the cement plugs reacted in the presence of YNPF and ENFG are broadly similar. The alteration is characterised by the development of a ‘chicken-wire-like’ network fabric of anastomosing calcium carbonated sealed microfractures similar to that seen in the experiments with gaseous CO2 (Plate 23). However, the degree of carbonation and alteration within this very narrow zone was much more intense with more complete replacement of the cement matrix by microcrystalline calcium carbonate and secondary amorphous silica (e.g. Plate 24). Within the altered cement, former air bubbles entrained within the original cement paste are largely infilled with coarse secondary calcite (Plate 25). Many of these are completely infilled, thereby almost completely destroying any macroporosity within the cement.
-
Plate 23 BSEM photomicrograph of the carbonated cement zone showing the ‘chicken-wire’ alteration fabric of anastomising interconnected microfractures and shrinkage cracks sealed by microcrystalline calcium carbonate (white), within a very fine matrix of microcrystalline calcite disseminated in an amorphous silica groundmass. ‘YNFP+Cl + supercritical CO2’, 40 days at 40°C under 80 bar pressure experiment. -
Plate 24 BSEM photomicrograph of the highly-altered matrix of the carbonated cement zone showing complete replacement of the CSH and CASH (and any partially-hydrated clinker phases) in the cement past by nano- to microcrystalline calcium carbonate (white), within a very fine matrix of amorphous silica (dull grey). Fine secondary cavities and microporosity (black) is also evident. ‘ENFG + supercritical CO2’, 40 days at 40°C under 80 bar pressure experiment.
-
Plate 25 BSEM photomicrograph of the carbonated cement zone showing spherical voids after air bubbles entrained in the cement paste are now largely filled by coarse secondary calcite. ‘YNFP+Cl + supercritical CO2’, 40 days at 40°C under 80 bar pressure experiment. -
Plate 26 BSEM photomicrograph showing the very sharply-defined main carbonation reaction front demarcating the inner region of partially-carbonated cement (left) from the outer reaction zone of intensely carbonated cement (right). The reaction front is defined by a narrow zone of intense precipitation of dense microcrystalline calcite, with a sharp ragged leading edge, and a more diffuse tail. ‘YNFP+Cl + supercritical CO2’, 40 days at 40°C under 80 bar pressure experiment. YNPF+Cl 40 days.
Detailed BSEM-EDXA observations show that the main carbonation front is characterised by a very sharp leading edge, which is very ragged and ‘fingers’ into the partially carbonated cement ahead (Plate 26). The trailing edge of this reaction front is characteristically diffuse, and over a distance of 0.1–0.5 mm it grades into highly altered cement characterised by the chicken-wire microfracturing and calcite mineralisation described above. The petrolographic features suggest that the processes of calcium carbonate precipitation observed at this reaction front are very complex and dynamic, and that calcium carbonate must be redissolving and redistributing within the anastomising network of calcite-mineralised veinlets behind the advancing reaction front.
The ‘dry supercritical CO2’ experiments did not display sharply-defined reaction fronts (Plate 22). The carbonation alteration was pervasive through the whole cement plug. However, BSEM-EDXA observations revealed that the carbonated cement fabric was characterised by the development of a ‘chicken-wire-like’ network fabric of anastomosing calcium carbonated sealed microfractures similar to that seen in the experiments with supercritical and gaseous CO2 experiments in the presence of an aqueous phase (Plate 27). However, in this case, little or no evidence of calcium carbonate precipitation was observed to seal air-bubbles that were entrained within the original cement paste. Consequently, much of the macroporosity in the cement plugs was preserved in these experiments.
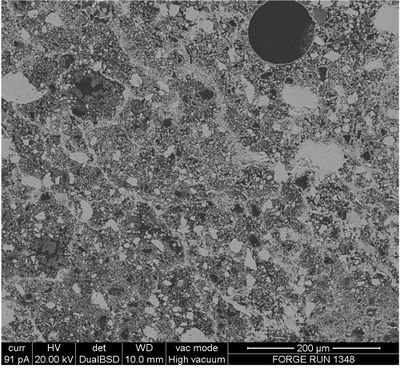
As in the experiments with gaseous CO2 in the presence of YNPF and ENFG, BSEM-EDXA analyses showed that the ‘YNFP+Cl + supercritical CO2’ and ‘ENFG + supercritical CO2’ experiments both demonstrated a concentration of Cl close to the leading edge of the main carbonation front (Figure 15). However, the distribution of Cl was found to be subtlety different, in that the Cl is concentrated ahead of the main calcium carbonate replacement front in these experiments, rather than behind the main reaction front. Also, no discrete Cl-bearing phase was found in the experiments with supercritical CO2. The concentration of Cl at the leading edge of the reaction front in these carbonation experiments accounts for the significant depletion of Cl- observed in the fluid. Although only a transient phase, the formation of this reaction product may potentially be important as a mechanism of retarding the migration of 36Cl from low- and intermediate-level radioactive waste.
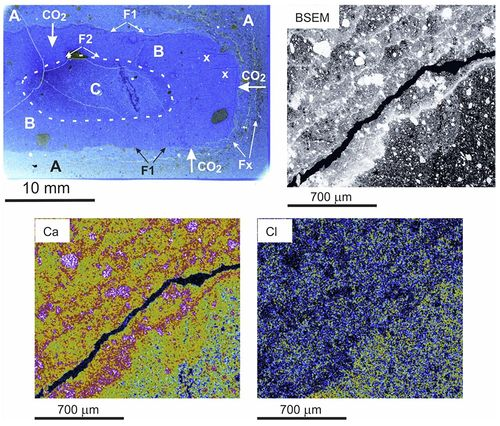
Carbonation with liquid CO2
The alteration characteristics of NRVB cement with supercritical CO2 in the presence of YNPF or ENFG are very similar. As with gaseous CO2 (see Surface alteration characteristics of the cement cores) and supercritical CO2 (see Carbonation with gaseous CO2), the reaction with liquid CO2 is demarcated by very sharp reaction fronts (Plate 28), which migrate deeper into the cement with time. In the case of the ‘YNPF + liquid CO2’ experiment, the cement sample appears to be strongly carbonated after 40 days, with only a small relict of partially reacted cement paste in the centre of the core (Plate 28). With ENFG the carbonation reaction front appears to be less advanced than with YNPF, although there is diffuse pervasive carbonation of the cement matrix throughout he whole sample (Plate 28). Transmitted light optical microscope observations show that there is an intermediate zone of of slightly more nano- to micro-porous cement developed ahead of the main carbonation front in the experiment with ENFG (Plate 28), similar to that see in the same experiment with supercritical CO2. This appears to be partially altered to very fine grained calcium carbonate but also partially leached of Ca in comparison to either the dense calcite-replaced outer reaction zone or the residual and partially altered cement in the core of the cement plugs.
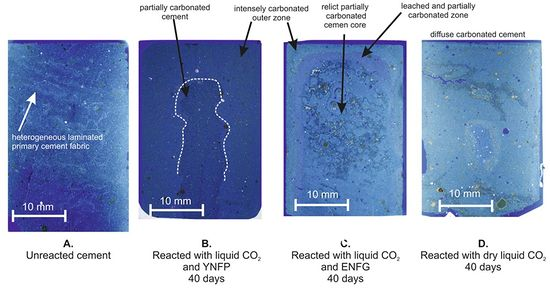
The alteration by dry liquid CO2 is similar to that observed with dry supercritical CO2. It did not produce a discrete reaction front. The experiment resulted in diffuse alteration to a highly-carbonated cement comprising a mixture of very fine grained calcium carbonate and secondary silica-rich material, and with finely disseminated iron oxide is produced (Plate 22). Within this fine-grained highly-carbonated matrix, irregular relicts of unreacted cement paste may be present. These unreacted relicts are heterogeneously distributed, and reflect the original heterogeneous character of the original fabric of the cement paste.
In thin section the petrographic fabrics of the alteration in the presence of the YNPF and ENFG aqueous fluids are very similar to those observed with supercritical CO2 and gaseous CO2. The alteration is characterised by the development of a ‘chicken-wire-like’ network fabric of anastomosing calcium carbonated sealed microfractures. However, the replacement of the carbonated cement paste by calcium carbonate was seen to be notably more intense with ENFG as the aqueous phase (Plate 29). The carbonated matrix of the cement is replaced by an intimate mixture of amorphous silica-rich material with finely dispersed nano- to micro-crystalline calcium carbonate. Many of the air bubbles that were entrained in the original cement paste are open and unminerlised, even in the intensely carbonated outer regions of the cement plugs (Plate 29).
-
Plate 29 BSEM photomicrograph showing the very sharply-defined main carbonation reaction front demarcating the inner region of partially-carbonated cement (dull grey) from the outer reaction zone of intensely carbonated cement (light grey). The reaction front is defined by a narrow zone of intense precipitation of dense microcrystalline calcite (see Plate 30), with a sharp ragged leading edge, and a more diffuse tail. ‘ENFG + liquid CO2’, 40 days at 20°C under 80 bar pressure experiment. -
Plate 30 BSEM photomicrograph showing detail of the very sharply-defined main carbonation reaction front. The reaction front is defined by a narrow zone of intense precipitation of dense microcrystalline calcite with fibrous calcium carbonate (possible aragonite) precipitated in the intensely carbonated cement behind the reaction front. ‘ENFG + liquid CO2’, 40 days at 20°C under 80 bar pressure experiment.
The main reaction front is defined by a narrow sharp front of microcrystalline calcium carbonate (probably calcite). However, in the experiment with ENPF fine fibrous needles of calcium carbonate — probably aragonite (based on morphological considerations) — is formed. This was not seen in the other experiments.
As with the experiments with supercritical CO2 and gaseous CO2, BSEM-EDXA observations indicate that Cl may be concentrated immediately ahead of the main reaction front in the 'ENFG + liquid CO2’ experiment. However, no discrete Cl-bearing phase could be differentiated.
References
- ↑ ALLEN, J R L. 1982. Sedimentary Structures, Their Character and Physical Basis. Vol. 2.: Elsevier Scientific Publications, Amsterdam.
- ↑ MILLS, P C. 1983. Genesis and diagnostic value of soft-sediment deformation structures — a review. Sedimentary Geology, 35, 83–104.
- ↑ CAREY, J W, WIGAND, M, CHIPERA, S J, WOLDEGABRIEL, G, PAWAR, R, LICHTNER, P C, WEHNER, S C, RAINES, M A, and GUTHRIE, J. 2007. Analysis and performance of oil well cement with 30 years of CO2 exposure from the SACROC Unit, West Texas, USA. International Journal of Greenhouse Gas Control, 1, 75–85.
- ↑ ROCHELLE, C A, MILODOWSKI, A E, SHI, J-Q, MUNOZ-MENDEZ, G, JACQUEMET, N, and LECOLIER, E. 2007. A review of the potential impact of CO2 on the integrity of well infrastructure for underground CO2 storage. British Geological Survey Commissioned Report, CR/07/204, 83p.
- ↑ Jump up to: 5.0 5.1 ROCHELLE, C A, MILODOWSKI, A E, LACINSKA, A, RICHARDSON, C, SHAW, R, TAYLOR, H, WAGNER, D, BATEMAN, K, LÉCOLIER, E, FERRER, N, LAMY, F, JACQUEMET, N, SHI, JI-Q, DURUCAN, S, and SYED, A S. 2009. JRAP-14: Reactions between CO2 and borehole infrastructure, report on laboratory experiments and modelling. CO2GeoNet project report for the European Commission, deliverable JRAP-14/3, 138p.